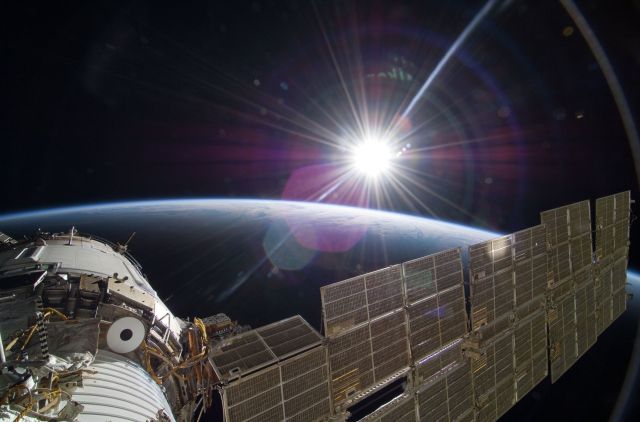
Paddy Neumann, a PhD student at the University of Sydney, has developed a new kind of ion space drive that has smashed NASA’s current fuel efficiency record. NASA’s High Power Electric Propulsion, or HiPEP system, allows 9,600 (+/-200) seconds of specific impulse. Neumann Drive has touched 14,690 (+/-2,000).
According to Australian newspaper Honi Soit, NASA’s HiPEP runs on Xenon gas, while the Neumann Drive can be powered on a number of different metals which produce ions when they are struck by an electric arc; the most efficient tested so far being magnesium. As it runs on metals commonly found in space junk, it could potentially be fuelled by recycling exhausted satellites, repurposing them into fresh fuel.
“Unlike current industry standard chemical propulsion devices, which operate through short, high-powered bursts of thrust and then coasting, Neumann’s drive runs on a continuous rhythm of short and light bursts, preserving the fuel source but requiring long-term missions,” write Joanna Connolly and Peter Walsh from Honi Soit.
In NASA’s HiPEP system, the Xenon gas is given an electrical charge. It is then electrically accelerated to a speed of about 30 km/second. When xenon ions are emitted at high speed, for example, as exhaust from a spacecraft, they push the spacecraft in the opposite direction. The ultimate speed a spacecraft can reach using ion thrust depends upon how much propellant it carries.
“Given the current cost of transporting fuel into space (exponential), and the ubiquity of space junk, the Neumann drive has huge commercial potential. It could vastly reduce the cost of space transportation, keep satellites in orbit for longer periods of time, and enable space travel of much greater distances, with Neumann suggesting the possibly of “Mars and back on a tank of fuel”,” writes the newspaper.
Neumann has applied for a patent for his invention, opening up the potential to commercialise the Drive down the track. To examine its performance under the conditions likely to be met in space, he is currently endurance-testing his Drive. His findings will be presented on September 30 at the 15th Australian Space Research Conference.
This Article (Australian Student’s Ion Space Drive Beats NASA’s Fuel Efficiency Record) is free and open source. You have permission to republish this article under a Creative Commons license with attribution to the author and AnonHQ.com.
I’m So proud to be an Australian and now the guy must watch his back because America is gonna kill him and make it look like an accident.
He should realise it online! then when america does kill him like they did tesla they will just supress it like they did with all of teslas stuff.
Citation: Shock wave for compression by Magnetic mirror holds promise for fusion in between positive and negative frequency swing in making fusion more realistic
In an elementary sense the physics of the thing is rather simple: the force of the shock wave produced by the fission-bomb explosion, is strong enough to compress the heavy hydrogen based target to fusion and, furthermore, the neutrons produced from the fusion lead to more fissions leading to the release of an overall high energy in the process. This is what one tries to do, quite unsuccessfully up to now, with the “inertial fusion” in the USA’s National Ignition Facility (NIF) with the laser beams.
Boeing has received a patent for, I kid you not, a laser-powered fusion-fission jet propulsion system. Boeing envisions that this system could replace both rocket and turbofan engines, powering everything from spacecraft to missiles to airplanes. The hot gases produced by the fusion are pushed out of a nozzle at the back of the engine, creating thrust—but that’s not all! One of the by-products of hydrogen fusion is lots of fast neutrons. In Boeing’s patented design, there is a shield around the fusion chamber that’s coated with a fissionable material (uranium-238 is one example given). The neutrons hit the fissionable material, causing a fission reaction that generates lots of heat. Finally, there’s some kind of heat exchanger system that takes the heat from the fission reaction and uses that heat (via a heated liquid or gas) to drive a turbine. This turbine generates the electricity that powers the lasers. Voilà: a fusion-fission rocket engine thing. Putting aside the difficulties of inertial confinement fusion (we’re nowhere near hitting the break-even point), it’s also a bit far-fetched to shoehorn all of these disparate and rather difficult-to-work-with technologies into a small chassis that hangs from the wing of a commercial airplane.
Given these thoughts, you would think that I would be enthusiastic about alternative fusion schemes. Yet, because I am vaguely aware of the challenges, and how robustly they are being addressed, I tend to greet alternative fusion schemes with some skepticism. So, it is with some interest and a bit of trepidation that I started examining new research on the Polywell fusion concept, where a group claims to have achieved grand new things in terms of plasma confinement.
But generally only elements above a certain mass are amenable to fission (these are called, appropriately enough, “fissile elements”). At the other end of the periodic table, lighter elements can be combined to heavier elements, and in doing so, become more stable. The very lightest element, hydrogen, can have its nuclei forced together to make a helium nucleus. This releases energy because the helium nucleus can exist in a more stable, lower energy ground state.
The kicker, though, is that it requires a great deal of energy to force the hydrogen nuclei close enough together for them to start fusing. Methods to achieve this can be divided into two classes: the “we crush solidified hydrogen using big-ass lasers” class, or the “we crush plasmas with big-ass magnetic fields” class.
Fusion, the energetic process that powers the Sun, is really a golden dream for unlimited and almost clean energy. Nuclear reactors generating electricity today operate on the principle of fission, or splitting elements apart. There are some elements—usually very heavy ones—that are inherently unstable. These elements fall apart to form different, more stable elements. A fission reactor coaxes these elements apart (usually by banging neutrons into them), and in doing so the elements release energy, which the reactor captures and uses.
As much as I love me some lasers, today we are only going to discuss magnetic fields. The basic idea is that, once the electrons are stripped from the nuclei, the hydrogen takes the form of a plasma. A plasma is basically a gas consisting of electrons zipping around at very high speeds and much more slowly drifting ions (positively charged nuclei). Like a gas, plasma has a pressure that is related to its temperature and density. Additionally, because a plasma consists of charged particles, it can be contained and compressed by magnetic fields.
The reason I mention this is that one can differentiate between the natural pressure of the plasma and the pressure applied by a magnetic field. The ratio of these two, called beta, is an important parameter in fusion reactors. Essentially, the efficiency of fusion goes up rapidly as beta approaches one, so magnetic compression aims to get beta as close to that level as possible. On the face of it, this seems simple enough: for the most efficient reaction, add more magnetic field, get more beta. Unfortunately, two things happen. First, the magnetic confinement tends to leak, so as you squeeze the plasma harder, it escapes. Second, as you squeeze the plasma, it becomes more turbulent and generates massive currents and fields that may destroy your apparatus. There are no simple solutions to either of these problems. There are, however, complicated solutions that have allowed tokamak scientists to reach beta values as high as 0.4, though 0.1 is a more typical value, and the ITER fusion megaproject is expected to operate at a beta of 0.03.
The Polywell concept, instead, tries to create a magnetic box to confine the plasma in place, which reduces the turbulence and solves many control problems. However, a true box is simply not possible. This is because the force applied by a magnetic field depends on the direction of motion of a charged particle, which causes the electrons and ions to travel in a corkscrew motion around magnetic field lines. At each corner of the magnetic field box, the magnetic field lines point outward away from the center of the box, so the plasma can spiral out of the box. The upshot is that the harder you squeeze, the quicker the plasma leaks out, leaving you with a low beta plasma.
But researchers quickly realized that if the plasma was dense enough—in other words, if it had a high beta—it would exclude the magnetic field lines, creating a sharp boundary between the plasma and the magnetic field. The sharp boundary acts like a mirror for charged particles, vastly slowing their rate of ion escape. This unfortunately creates a chicken and egg scenario: if you have a high beta plasma, a Polywell design will keep it confined at high beta. But first, you must have a high beta plasma
One intelligent way is by laser compression between positive and negative frequencies of resonance as the waves are shifted between corresponding negative and positive wave lengths of alternative compression and expansion in between a neutral domain such a possibility could be realized. This device works by bouncing microwaves around inside a conical cavity that swings between positive and negative frequencies of typical laser tuned nozzles .According to him, the taper of the cavity creates a change in the group velocity of the microwaves as they move from one end to the other, which leads to an unbalanced force, which then translates into a thrust. If it worked, the EMDrive would be a propulsion method unlike any other, requiring no propellant to produce thrust. There is, of course, a flaw in this idea. The design instantly violates the principle of conservation of momentum. This states the total momentum (mass x velocity) of objects in a system must remain the same and is linked to Newton’s Third Law. Essentially, for an object to accelerate in one direction, there must be an equal force directed the opposite way. In the case of engines, this usually means firing out particles (such as propellant) or radiation.But uniflow directional thrust with laser has already been invented.
Main experimental results.
Amplification factor as function of seed chirp rate and pump energy
Electron thermal velocity distribution maps with superimposition of the seed amplitude (solid line) for (a) positive chirp, (b) negative chirp.
The experimental scientists involved have done their jobs to the best of their ability, having tested a hypothesis—albeit a spectacularly unlikely one—and reported their results. These scientists aren’t actually claiming to have invented a warp drive or to have broken the laws of physics. All they’re saying at the moment is that they’ve found something odd and unexplained that might be something new but is likely an experimental artefact that needs further study. The panoply of clickbait headlines and poorly researched articles on the topic are doing something of a disservice to their scientific integrity by claiming otherwise.
Ref:
1)Directional ejection of laser beams using Dirac cone ring emissions out of Leon Cooper electron spin dynamics: http://www.hawking.org.uk – 00351040
2)Laser squeezing in photon domains by increasing and decreasing the frequency and dampness between clockwise and anticlockwise dynamics along converging and diverging squeezing – 00347962
3) Very important Citation: Cooper electron transition between magnetic and electricfield cone connection between spiraling magneticfield and liner electric field phase transition may contribute to Mott Transition phase in between temperature variation – 00352659
References
1. Zetta-Exawatt Science and Technology. Eur. Phys. J. ST. 223, 979–1242 (2014).
2. WHITEBOOK ELI Extreme Light Infrastructure, Science and Technology with Ultra-Intense Lasers (CNRS, Paris, 2011).
3. Strickland D. & Mourou G. Compression of amplified chirped optical pulses. Opt. comm. 56, 219 (1985).
4. Kruer W. The Physics of Laser Plasma Interaction (Addison-Wesley, Reading, MA, 1988).
5. Malkin V. M., Shvets G. & Fisch N. J. Fast compression of laser beams to highly overcritical powers. Phys. Rev. Lett. 82, 4448 (1999).
6. Ping Y., Geltner I., Fisch N. J., Shvets G. & Suckewer S. Demonstration of ultrashort laser pulse amplification in plasmas by a counterpropagating pumping beam. Phys. Rev. E 62, R4532 (2000). [PubMed]
7. Ping Y., Geltner I., Morozov A., Fisch N. J. & Suckewer S. Raman amplification of ultrashort laser pulses in microcapillary plasmas. Phys. Rev. E 66, 046401 (2002). [PubMed]
8. Ping Y., Geltner I. & Suckewer S. Raman backscattering and amplification in a gas jet plasma. Phys. Rev. E 67, 016401 (2003). [PubMed]
9. Ping Y., Cheng W., Suckewer S., Clark D. S. & Fisch N. J. Amplification of ultrashort laser pulses by a resonant Raman scheme in a gas-jet plasma. Phys. Rev. Lett. 92, 175007 (2004). [PubMed]
10. Balakin A. A. et al. . Laser pulse amplification upon Raman backscattering in plasma produced in dielectric capillaries. JETP Lett. 80, 12 (2004).
11. Cheng W. et al. . Reaching the nonlinear regime of Raman amplification of ultrashort laser pulses. Phys. Rev. Lett. 94, 045003 (2005). [PubMed]
12. Ren J., Cheng W., Li S. & Suckewer S. A new method for generating ultraintense and ultrashort laser pulses. Nat. Phys. 3, 732 (2007).
13. Vieux G. et al. . Chirped pulse Raman amplification in plasma. New J. Phys. 13, 063042 (2011).
14. Turnbull D., Li S., Morozov A. & Suckewer S. Possible origins of a time-resolved frequency shift in Raman plasma amplifiers. Phys. Plasmas 19, 073103 (2012).
15. Shvets G., Fisch N. J., Pukhov A. & Meyer-ter Vehn J. Superradiant amplification of an ultrashort laser pulse in a plasma by a counterpropagating pump. Phys. Rev. Lett. 81, 4879 (1998).
16. Malkin V. M., Shvets G. & Fisch N. J. Ultra-powerful compact amplifiers for short laser pulses. Phys. Plasmas 7, 2232 (2000).
17. Malkin V. M. & Fisch N. J. Quasitransient backward Raman amplification of powerful laser pulses in dense plasmas with multicharged ions. Phys. Plasmas 17, 073109 (2010).
18. Trines R. M. G. M. et al. . Simulations of efficient Raman amplification into the multipetawatt regime. Nat. Phys. 7, 87 (2011).
19. Robinson P. A. Nonlinear wave collapse and strong turbulence. Rev. Mod. Phys. 69, 507 (1997).
20. Bohm D. & Gross E. P. Theory of plasma oscillations. a. Origin of medium-like behavior. Phys. Rev. 75, 1851 (1949).
21. Coffey T. P. Breaking of large amplitude plasma oscillations. Phys. Fluids 14, 1402 (1971).
22. Ren J. et al. . A compact double-pass Raman backscattering amplifier/compressor. Phys. Plasmas 15, 056702 (2008).
23. Yampolsky N. A. et al. . Demonstration of detuning and wavebreaking effects on Raman amplification efficiency in plasma. Phys. Plasmas 15, 113104 (2008).
24. Ersfeld B., Farmer J., Raj G. & Jaroszynski D. A. The role of absorption in Raman amplification in warm plasma. Phys. Plasmas 17, 083301 (2010).
25. Farmer J. P., Ersfeld B. & Jaroszynski D. A. Raman amplification in plasma: Wavebreaking and heating effects. Phys. Plasmas 17, 113301 (2010).
26. Yampolsky N. A. & Fisch N. J., Limiting effects on laser compression by resonant backward Raman scattering in modern experiments. Phys. Plasmas 18, 056711 (2011).
27. Turnbull D., Li S., Morozov A. & Suckewer S. Simultaneous stimulated Raman, Brillouin, and electron-acoustic scattering reveals a potential saturation mechanism in Raman plasma amplifiers. Phys. Plasmas 19, 083109 (2012).
28. Butler A., Spence D. J. & Hooker S. M. Guiding of high-intensity laser pulses with a hydrogen-filled capillary discharge waveguide. Phys. Rev. Lett. 89, 185003 (2002). [PubMed]
29. Ersfeld B. & Jaroszynski D. A. Superradiant linear Raman amplification in plasma using a chirped pump pulse. Phys. Rev. Lett. 95, 165002 (2005). [PubMed]
30. Nuter R. & Tikhonchuk V. Prepulse suppression and optimization of backward Raman amplification with a chirped pump laser beam. Phys. Rev. E 87, 043109 (2013). [PubMed]
31. Berger R. L., Clark D. S., Solodov A. A., Valeo E. J. & Fisch N. J. Inverse bremsstrahlung stabilization of noise in the generation of ultrashort intense pulses by backward Raman amplification. Phys. Plasmas 11, 1931 (2004).
32. Jaroszynski D. A. et al. . The Strathclyde Terahertz to Optical Pulse Source (tops). Nucl. Instr. and Meth. in Phy. Res. A 445, 317 (2000).
33. Abuazoum S. et al. . Linearly tapered discharge capillary waveguides as a medium for a laser plasma wakefield accelerator. Appl. Phys. Lett. 100, 014106 (2012).
34. Kline J. L. et al. . Different kλD regimes for nonlinear effects on Langmuir waves. Phys. Plasmas 13, 055906 (2006).
35. Morales G. J. & O’Neil T. M. Nonlinear frequency shift of an electron plasma wave. Phys. Rev. Lett. 28, 417 (1972).
36. Lindberg R. R., Charman A. E. & Wurtele J. S. Reduced kinetic description of weakly-driven plasma waves. Phys. Plasmas 15, 055911 (2008).
37. Toroker Z., Malkin V. M. & Fisch N. J. Seed laser chirping for enhanced backward Raman amplification in plasmas. Phys. Rev. Lett. 109, 085003 (2012). [PubMed]
38. Hur M. S., Penn G., Wurtele J. S. & Lindberg R. Slowly varying envelope kinetic simulations of pulse amplification by Raman backscattering. Phys. Plasmas 11, 5204 (2004).
39. Chapman T. et al. . Driven spatially autoresonant stimulated Raman scattering in the kinetic regime. Phys. Rev. Lett. 108, 145003 (2012). [PubMed]
40. Malkin V. & Fisch N. Key plasma parameters for resonant backward Raman amplification in plasma. Eur. Phys. J. ST 223, 1157 (2014).
41. Wiggins S. M. et al. . Note: Femtosecond laser micromachining of straight and linearly tapered capillary discharge waveguides. Rev. Sci. Instrum. 82, 096104 (2011). [PubMed]
42. DeLong K. W., Kohler B., Wilson K., Fittinghoff D. N. & Trebino R. Pulse retrieval in frequency-resolved optical gating based on the method of generalized projections. Opt. Lett. 19, 2152 (1994). [PubMed]
43. Swamp Optics website http://www.swampoptics.com. Date of access: 17/05/2015.
Very important Citation: Cooper electron transition between magnetic and electricfield cone connection between spiraling magneticfield and liner electric field phase transition may contribute to Mott Transition phase in between temperature variationhttp://www.hawking.org.uk
Very important Citation: Cooper electron transition between magnetic and electricfield cone connection between spiraling magneticfield and liner electric field phase transition may contribute to Mott Transition phase in between temperature variation – 00352659
Disqus reference this is Case #450644
A Mott transition is a metal-nonmetal transition in condensed matter. Due to electric field screening the potential energy becomes much more sharply (exponentially) peaked around the equilibrium position of the atom and electrons become localized and can no longer conduct a current. The monolayer form is normally an insulator, but when stretched, it can become electrically conductive operative under Seebeck effect producing a Cooper cooling Mott valley a stretch from neutral plane towards left or right vary the magnetic and electrifield intensity as leading or lagging factor.
In between spin opposite and colascence there must be magneticfield twisters that swing between spiral and linear ,when the spirality is more leading to increase in magneticfield and linearity contributing to more electricfield which may be called an electric screening
Previous X-ray observations revealed that the Circinus X-1’s neutron star has a relatively small magnetic field. “General theory holds neutron stars are born with a large magnetic field,” says Heinz. “This newly minted neutron star has a field much smaller than expected.” When a once-massive star explodes as a supernova, it creates either a black hole or a neutron star, a condensed, rapidly-spinning cinder with extraordinary gravitational pull. The unusual elliptical orbit on which these two stars swing around each other is exactly what you would expect for a very young X-ray binary. In cool stars, such as the Sun, magnetic fields are generated by convection in the outer portion of the star. However, there is no convection in the outer layers of massive star, so there is no support for a magnetic dynamo. Nevertheless, approximately 10 per cent of massive stars have strong magnetic fields. A phase transition from an insulating to a metallic state is induced by driving an electrical current through the system; One system that unites the two is found in superconductors, certain materials that conduct electricity perfectly when cooled to very low temperatures. Magnetic fields penetrate the superconducting material in the form of tiny filaments called vortices, which control the electronic and magnetic properties of the materials. a general understanding of out-of-equilibrium physics.
Scientists announced the first observation of a dynamic vortex Mott transition, which experimentally connects the worlds of quantum mechanics and classical physics and could shed light on the poorly understood world of non-equilibrium physics.
The discovery experimentally connects the worlds of classical and quantum mechanics and illuminates the mysterious nature of the Mott transition. It also could shed light on non-equilibrium physics, which is poorly understood but governs most of what occurs in our world. Two explanations have been proposed for the origin of massive star magnetic fields, both variants on the idea of a so-called “fossil” magnetic field, which is generated at some point in the star’s past and then locked in to the star’s outer portion.
The first hypothesis is that the magnetic field is generated while the star is being formed; the second is that the magnetic field originates in dynamos driven by the violent mixing of stellar plasma when the two stars in a close binary merge.
The horizontal and vertical alignment between electric and magnetic field vector contributing the speed of spinning maximum at pi/2 phase and minimum at zero phase the speed varied along such an interaction.
Sankaravelayudhan Nandakumar on behalf of Calendron Lab, Oxford astrophysics research center working under Simon Hooker as guided by Hon .Stephen Hawking ,Applied mathematical algorithmic research center at Cambridge University.
This means that Cooper electron transition between magnetic and electricfield cone connection between spiraling magneticfield and liner electric field phase transition may contribute to Mott Trnasition phase in between temperature variation.
Citation: Strange Cooper electron as it swings and wobbles along ellipsoidal oblivion forming a deviation from classical quantum mechanics http://www.hawking.org.uk
Ref:
1)Directional ejection of laser beams using Dirac cone ring emissions out of Leon Cooper electron spin dynamics: http://www.hawking.org.uk – 00351040
2)Laser squeezing in photon domains by increasing and decreasing the frequency and dampness between clockwise and anticlockwise dynamics along converging and diverging squeezing – 00347962
Strange Cooper electron as it swing and wobble along ellipsoidal oblivion the speed is varied in spin axis and erratic leading to repulsive attractive energy shift in between a deviation from quantum mechanical theory where electron absorb the energy when shifted from lower orbit to higher orbit emit quantum energy when shifted from higher to lower energy which become complex at these complex combinational planes. This is also application of varying speed Blackholes says Sankaravelayudhan Nadakumar on behalf of Applied Mathematical research Center Cambridge. Electron spins slowly enough; it won’t repel its meal as much. In the end, a slow-spinning Electron calls for an attraction can gobble up more matter waves than a fast spinner. The fast spinning electron repulses the matter wave.
The future of electronics lies in optical control of electron flows. That would enable data processing operations to be performed at frequencies equivalent to the rate of oscillation of visible light as it circulate along ellipsoidal orbit. This is also applicable in case of Mercury orbital motion of solar system. Not alone this there must be conversion emission from half spin fermions leading and lagging full spin boson emission and formation of complex binary stars emission can also be detected.
Sankaravelayudhan Nandakumar on behalf of Calendron Lab, Oxford astrophysics research center working under Simon Hooker as guided by Hon .Stephen Hawking ,Applied mathematical algorithmic research center at Cambridge University.
Copy to crick